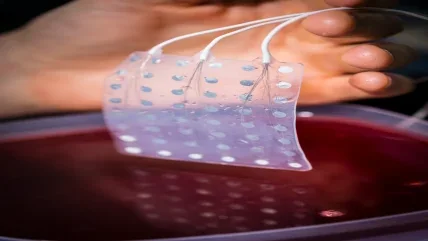
They have helped save and improve countless lives, but the devices currently used to map brains for resection still look disconcertingly like they have been cut out from a Sunday newspaper’s brainteaser page – as if Hannibal Lecter had his own pulsating spin on Sudoku. Electrocorticography (ECoG) grids, as these tools are called, consist of numbered electrical contacts spaced 1cm apart. Placed directly onto the surface of a patient’s brain, they’re used to detect and isolate the locations of different cognitive domains or functions. Surgery teams employ them in working out how to remove brain tumours without permanently damaging faculties like language, memory or motor control. The numbers – or sometimes letters – are used as reference points to note approximately where those functions reside in individual patients.
Approximately is the operative word there. The 1cm2 separating each sensor equates to a very low-resolution image, so neurosurgeons have to work with a linear extrapolation of the irregular boundaries between, for instance, a patient’s motor and sensory cortexes. The established practice is to incorporate 5–10mm resection margins to avoid causing off-target damage, but that can prevent surgeons from fully removing tumours – and still leaves room for error.
It is not a new problem. Today’s ECoG grids are manufactured much as they were when they were first used – all the way back in the 1950s. Millimetre-scale electrodes are pressed into a layer of biocompatible silicone approximately 1mm thick and then hand soldered to electrical wires. For all the technological developments of the past 70 years, human hands remain roughly as dextrous as they always have been, so significantly improving the resolution offered by ECoG grids means rethinking the entire manufacturing process. That is exactly what Shadi Dayeh, Ahmed Raslan and their team have done. Their next-generation arrays provide electrocorticography for our flat-screen, high-definition age, in more ways than one.
Combine to build
As a neurosurgeon that specialises in resecting tumours from functional brain regions, Raslan, associate professor of neurological surgery at Oregon Health and Science University, has spent a career pushing up against the limitations of handmade ECoG grids. When he saw Dayeh, professor of electrical and computer engineering at the University of California San Diego, presenting an abstract about high-resolution sensor arrays, he knew he had found the “unicorn” that could finally help him overcome them. Together, the duo has developed the idea to incorporate 1,024 or 2,048 ECoG electrodes in 3x3cm and 6x6cm grids just 7μ thick.
“It’s very similar to looking at the sun but using a 100 times more powerful telescope,” says Raslan, referring to the 3cm grid that fits 100 electrodes. Today’s clinical equivalents can only manage one. “You start seeing things that you didn’t know existed.”
There are plenty more metaphors where that came from. Raslan is almost as good at giving poetic descriptions of the brain as he is at cutting into it. He explains what he has learned about the border between the parts of the brain responsible for movement and sensation, a boundary usually identified with a specific fold in the brain called the central sulcus, with reference to the difficulty of drawing a line between the land and the sea. Without next-generation ECoGs, the observation, and the improvements it could mean for his practice, may not have been possible.
“It was clearly evident to us from this very high resolution that the central sulcus may not always be the functional boundary; the functional boundary is different from one human to another,” says Raslan. “And it’s not always in front, behind or parallel to the central sulcus: it can cross. It doesn’t have a linear dimension. It’s like the shoreline.”
Imagine a world map with every border and coast flattened into straight lines and you will have some idea of what surgeons using traditional ECoG grids are navigating by. “It’s very important,” explains Raslan, “because, generally speaking, you want to perform resection where there’s no motor function, but if the boundary that you’re using to delineate that area is incorrect, you can make the wrong decision. That’s one of the very first things we learned.
“Now, you can have basically a resection margin of half a millimetre,” Dayeh adds. “You can look at the curvilinear boundary of the tumour, and only resect the tumour and preserve function.” More than just helping surgery teams differentiate brain regions, that enables fine distinctions within each functional area. “We can really observe these boundaries at the millimetre scale, and even define correlates for finger movements.”
Equivalent levels of precision are currently attainable in research contexts thanks to Blackrock Neurotech’s Utah Array. However, at scarcely 5mm2, that is not the best device for getting the wider understanding often needed in planning a resection. It is also an expensive, difficult to manufacture technology that only works by penetrating the surface of the brain – which makes it hard to use in the middle of an operation.
“Conventionally there have been trade-offs that made the Utah Array a research-grade device and the ECoG grid a clinical grade device,” explains Raslan. “If you want to record a small area of the surface of the brain, or from individual cells, you have to penetrate; and you can either get a very high resolution or a large surface area coverage, but you can’t get both.” Until now. “In essence, this grid brings the research capabilities that we had previously available in the Utah Array to the clinical space. It turns the operating room into a very advanced research laboratory.”
The crystal ball
That would not be possible if not for the years of near-obsessive attention that Dayeh’s team at UCSD has given to material and microfabrication. Their idea began with crystals of PEDOT embedded in polyimide, but it reached its fruition with platinum nanorods and parylene C.
PEDOT, a one-dimensional crystal, was originally chosen because of its ability to sense volumetrically rather than simply on its surface. That lowers electromechanical impedance and increases sensitivity, which is important for moving from millimetre to micron scale ECoG sensors without introducing extra noise to their readings. PEDOT, though, is not the most stable material, so the team spent a long time fabricating metal nanostructures that could mechanically lock it in place. Eventually, Dayeh was struck by the fact that the nanostructures his graduate students were developing were themselves capable of volumetric sensing. All the work that had been done to make them suitable for securing PEDOT had made the PEDOT unnecessary. As platinum – the standard material for clinical electrodes – is the most biocompatible metal conductor, it was the obvious one to use for these PEDOT-free sensors. Using a dealloying technique modified from an old Nature paper, the team created high-surface area nanorods with a pure, stable and crystalline platinum ideal for interacting with tissue.
Importantly, unlike most approaches to fabricating nanostructures, that dealloying technique can happen at relatively low temperatures, which means it is compatible with parylene C, a material best known in the medical device industry as a coating for implants (including the Utah Array’s needles).
On top of the biocompatibility that suits it to that job, parylene C is soft and pliable enough to conform and move with the surface of the brain without obscuring it. “You can deposit it at thicknesses that are less than 10μ, and at those thicknesses, it becomes conformal to the brain curvature and compliant to the brain movements,” says Dayeh. “Because it’s a thin sheet that the brain feels is so light, it won’t have a biofouling reaction against it, and therefore, you can record with very high fidelity and isolate the activity in local brain regions because the electrical contact is always in intimate contact with the surface of the brain.”
That is helped further by the perfusion holes etched in the parylene C, which prevent spinal fluid building up beneath the grid. “That facilitates the grid to stick to the surface of the brain,” explains Raslan. “And not only that – you’re also able to send electrical current through the holes, so we could use a clinical stimulator device. The presence of the grid on the surface of the brain does not impede any of the normal clinical activity.”
Perhaps most impressively, it’s even possible to incorporate flaps into the parylene C, meaning surgeons can monitor brain activity and function, peel back the flap and perform the resection before folding the flap back over to continue recording and make an informed decision over what to do next.
It is nothing like the current practice, which usually involves a neurophysiologist copying ECoG readings of different brain regions from a computer screen onto a printed map. “The surgeon will then try to project based on the context or put a sterile paper on the surface of the brain to mark these regions and match them to the grid,” Dayeh explains. “There’s a lot of back and forth and shouting – and that’s a big theme already in the OR.”
But there are other difficulties; not least the challenge of displaying and interpreting data from a grid of 1,000 channels, as opposed to a few tens. Dayeh’s team is working to smooth the transition with real-time displays of ECoG readings that can zoom in or highlight certain channels to present surgical teams with only the information they need at any given moment in a procedure. “It would really simplify the procedure a lot if we’re able to have a real-time display either directly from the surface of the brain using light emitting diodes, or on a computer screen next to the surgeon, where he could visibly see the regions and correlate them with the anatomy on the brain surface,” Dayeh notes.
That’s not the only intersection between Dayeh and Raslan’s next-generation ECoGs and high-resolution screens. For Dayeh in particular, making use of modern display technology is key to achieving the holy grail and bringing effective treatments like resections to more people – not just by making procedures easier for surgical teams, but also as a way of manufacturing ECoGs as quickly and affordably as possible. Today’s ubiquitous screens are made using a low-temperature process that just so happens to be ideal for working with parylene C.
“The problem of the temperature has been solved by the display industry, so why don’t we learn from them and apply it to the brain?” asks Dayeh. “They’re doing displays for flexible phones on glass substrates at low temperatures, and, because there are very big screens, production on glass exceeds 2m in height and width, so you can make hundreds of grids on one plate. It’s really scalable, and it can be done at low cost.”
It is a convincing case. Dayeh and Raslan’s new ECoGs promise to improve upon the capabilities of the Utah Array while being much better suited to use in the clinic, thanks largely to manufacturing techniques that are already well established for producing the screens that our brains so love to be distracted by. Clinical trials are ahead, and the team still needs to work out how best to transfer and communicate data from so many channels, but the potential is staggering.
“It’s a platform technology,” says Raslan. “People will develop uses that we haven’t found. You could use it for brain stimulation, for brain-computer interfaces, for rehabilitation, or for epilepsy detection. It’s really a device that connects you with the brain in an unprecedented way.”
100
The number of electrodes that fit in the newly developed 3cm grid – today’s clinical equivalents can only manage one.
University of California San Diego